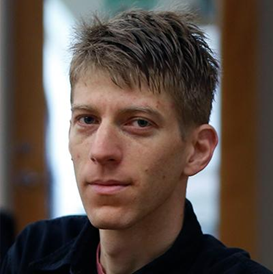
Synthetic Bioelectrical Materials
Adam E. Cohen, Harvard University
Project Summary/Abstract. Approved for public release
Membrane voltage is unique among cellular signaling modalities for its ability to encode information on the sub-millisecond timescale, and to propagate signals within and between cells at speeds faster than 100 m/s. It is these attributes which make voltage a suitable substrate for conveying signals where precise timing and long-range correlations are important, e.g. encoding information in the brain or synchronizing contraction in the heart. I propose to develop an engineering discipline around synthetic bioelectrical materials: to create genetically, structurally, and compositionally engineered artificial tissues, where every ion channel and transporter is known and understood. By taking a bio-inspired engineering approach, we aim to learn about the fundamental requirements for information processing in active cellular matter, and to create cellular biomaterials with novel sensing, pattern forming, and computational properties. This work will span cell biology, electrical engineering, condensed matter physics, and nonlinear dynamics.
While this ambition may seem farfetched—and certainly some aims will require years to achieve—the core tools are now in place. The detailed functional properties of many individual ion channels are known. It is now routine to express exogenous ion channels in mammalian cells. And my lab has developed sophisticated instrumentation for optical interfacing with light-gated ion channels and with fluorescent voltage indicators.
While we understand the individual ion channel components, startling and unanticipated effects can arise when millions of electrically active cells interact. This emergence of collective phenomena has a parallel to condensed matter physics, where the properties of a bulk material may differ dramatically from those of the unit cell. Cellular syncytia can support collective electrical waves, electrical domain boundaries, and electrical defects. Under some conditions these dynamics can lead to bistability or chaos (as happens, often tragically, in cardiac arrhythmias). We have predicted theoretically that electrically coupled cellular syncytia can produce Turing-like spatial patterns, though this effect has not yet been observed.
What are the rules that govern collective behavior of electrically active cells? When will the cells show stable patterns, bistability, waves, or chaos? How do these cells respond to perturbations—what are the excited states of an electrically active cellular monolayer? These are some of the basic research questions we will address.
Idealized cellular automata have been a favorite model of computer scientists and mathematicians, but have existed in a largely theoretical domain. Cellular automata been mathematically shown to be capable of forming Turing complete computational systems, i.e. computers. Can we implement such a system out of real electrically active cells? The discipline of working with real cells, and of grappling with disorder and noise, will undoubtedly shed insights into which features of these systems are robust and which require fine tuning. These effort will illuminate the physical underpinnings of biological computation, and may ultimately lead to a new class of biological computers.
Engineered bioelectrical systems offer the promise of interesting uses, though specific applications are outside the scope of this proposal. We now understand the molecular basis for our senses of smell, sight, touch, and taste, in sufficient detail to recreate these sensory modalities in engineered cells, coupled to bioelectrical excitations. This capability opens the possibility, for instance, to amplify signals from a few odorant receptors to a macroscopic bioelectrical impulse. We also know how to turn bioelectrical impulses into optical, electrical, chemical or mechanical outputs. These capabilities could ultimately lead to fast, highly specific and ultra-sensitive chemical detectors with built-in logic and signal processing.